In the TWIGA proposal, we included one technology that was at the lowest Technology Readiness Level (TRL1). This was a cost-effective detector of environmental neutrons. This blog is a bit technical but, basically, we want to know how wet our soils are, at a scale that is comparable to that observed by satellites. Soil moisture is the key variable that controls the redistribution of precipitation on the surface between evaporation, run-off, and groundwater recharge. Traditional soil moisture measurements cover about 20 cm2, while the smallest pixels in satellite images cover a hundred square meters or more. So if we could measure soil moisture at a larger scale, we would be able to calibrate satellite products directly and dynamically. Neutrons may help to do exactly that.
Cosmic rays continuously bombard the Earth, causing cascades of high-energy sub-atomic particles that hit nuclei, that burst apart with many different sub-atomic parts, etc. One result is a very thin “gas” of neutrons, some of which are very energetic (fast neutrons). When these fast neutrons bounce into nuclei, they lose energy and become slow or thermalized neutrons. Fast and slow neutrons are in a dynamic equilibrium. The level of this equilibrium depends on the chances that a neutron bounces into a nucleus. It so happens that hydrogen has a relatively large cross-section for fast-moving neutrons, which means that hydrogen slows down fast neutrons very efficiently. This has been known for some time. Actually, the first experimental nuclear reactions were using this phenomenon. Otto Hahn, Lise Meitner, and Fritz Straßmann used this technology in the 1930s to discover the first splitting of the atom.


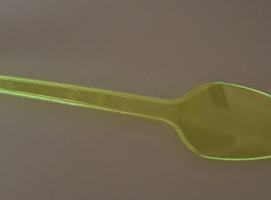
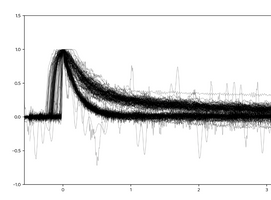
This all seems very far removed from any practical applications in the environmental sciences. But in 1966 [!], Hendrick and Edge[1] discovered that the ratio between fast and slow neutrons in our environment depends on how wet this environment is. Water is relatively hydrogen-rich and slows down the fast neutrons that are constantly being produced by incoming cosmic rays. So the higher the ratio between fast and slow neutrons, the drier this environment is. The nice thing is that this is very robust and does not depend on a very local measure of soil moisture but, roughly, on the soil moisture in a radius of about 300 m from the measurement. This, in turn, allows us to directly link satellite-based estimates of soil moisture, which typically have footprints of tens to hundreds of meters, with ground observations. It is not trivial to find average soil moisture contents at such scales by traditional means[2].
Hydrologists have known this for some time and have used instruments to measure the slow/fast ratios. The most efficient way to “catch” thermal neutrons is with Helium-3 (3He), as used in the COSMOS instrument (COsmic-ray Soil Moisture Observing System[3]). This consists of thermal neutron detectors, one surrounded by PVC, which slows down fast neutrons, just as the paraffin did in the lab. So one detector just registers thermals neutrons and the other detects the sum of neutral and fast neutrons. This is a very robust measurement device but the costs are high. Including power supply and telemetry, the set-up quickly goes in the direction of $ 40,000. 3He is an expensive compound and it is no surprise that only five COSMOS sensors can be found in Africa, of which three in South Africa
Enters the $100 muon detector[4]. A muon is a sub-atomic particle associated with very high-energy cosmic rays hitting the Earth’s atmosphere. These are very interesting to the astronomy community. Cosmic rays can come from very far away and sometimes have extremely high energies. Imagine a sub-atomic particle having the same kinetic energy as a tennis ball at over 100 km/h! In order to measure these particles, Spencer Axani at MIT developed an Arduino-based muon sensor for $100. From here to a cost-efficient neutron detector is just a small step, or so we hoped.
Basically, the muon detector uses a special piece of plastic that absorbs muons, at which point it produces photons in the process. This piece of plastic, called a scintillator, is monitored by a very sensitive light detector, called a Silicon Photon Multiplier (SiPM). A SiPm can detect individual photons with a 40% efficiency. All that there was left to do at TRL1 was to replace the muon-sensitive plastic with something that can detect neutrons and produce photons. Such detectors exist and are typically using isotopically enriched lithium-6 (6Li) or boron-10 (10B), and are used to detect smuggling of nuclear devices and similar things. When a neutron interacts with 10B, an alpha particle is produced that in turn produces photons when it interacts with other atoms. Unfortunately, such enriched elements are relatively expensive. However, for environmental applications, we could simply live with lower efficiency and increase the size of the instrument. For example, 20% of naturally occurring boron is the desired 10B. What if we simply make a somewhat bigger instrument at a much lower cost of, say, $200? This is what co-author Edward van Amelrooy has been working on for some months now.
The basic approach so far has been to have a thin layer of paint that contains natural boron and zinc-sulfide/silver pigments (ZnS-Ag). ZnS-Ag produces a lot of photons when hit by an alpha particle. The photons then have to be guided to a SiPM and registered. This is done by wrapping a reflecting layer, coated with this paint, around a piece of wavelength-shifting plastic. The ZnS-Ag pigments produce a blue light when hit by an alpha particle. When the blue light enters the plastic and interacts with a pigment that shifts the light down to green, the photon will be guided within the plastic, like a waveguide.
Instead of an ice-cream spoon, a rod of similar plastic has been used that is surrounded by a B-ZnS-Ag paint. At the end of the rod, a SiPM was attached to capture any photons that came out of the end of the rod. OK, you may say, this is all very simple and straightforward (thanks for staying with us for so long!) but, unfortunately, neutrons are not the only particles around that interact with ZnS. Ubiquitous gamma rays also cause a lot of photons to be produced. So, after some early excitement about possible neutron detection, a test at the TU Delft nuclear facility showed that the set-up produced many counts near gamma-ray sources. Typically, rather complicated and fast electronics and associated software filters are needed to distinguish between different particles such as gamma-rays and neutrons.
Last weekend (25-26 October 2020), a large number of signals were registered and superimposed, as shown in Figure 4. These measurements suggest that there is a statistically significant difference between the signal from two different processes that would be easily distinguished through the software on, say, an Arduino. The hypothesis is that the quickly declining signal comes from gamma rays and the more slowly declining signal from neutrons. This week, Edward will bike from his home to the nuclear reactor at the TU Delft campus to expose the sensor to different radiation sources to see if these differences are indeed what distinguishes gamma-rays from neutrons… fingers are crossed!
The ‘T’ in TWIGA stands for ‘Transforming’. Having 100 or more COSMOS instruments in Africa would indeed be transformative and make it a continent with the best thinkable soil moisture satellite calibration infrastructure.
Written by: Nick van de Giesen, Edward van Amelrooy
TUDELFT